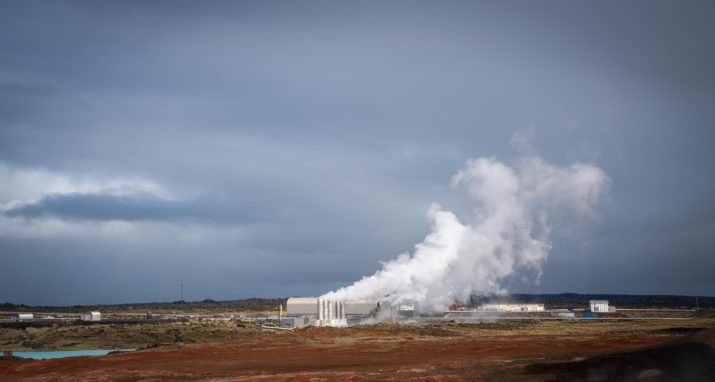
Geothermal Heating and Cooling Networks for Green and Livable Urban Transformations – Part I
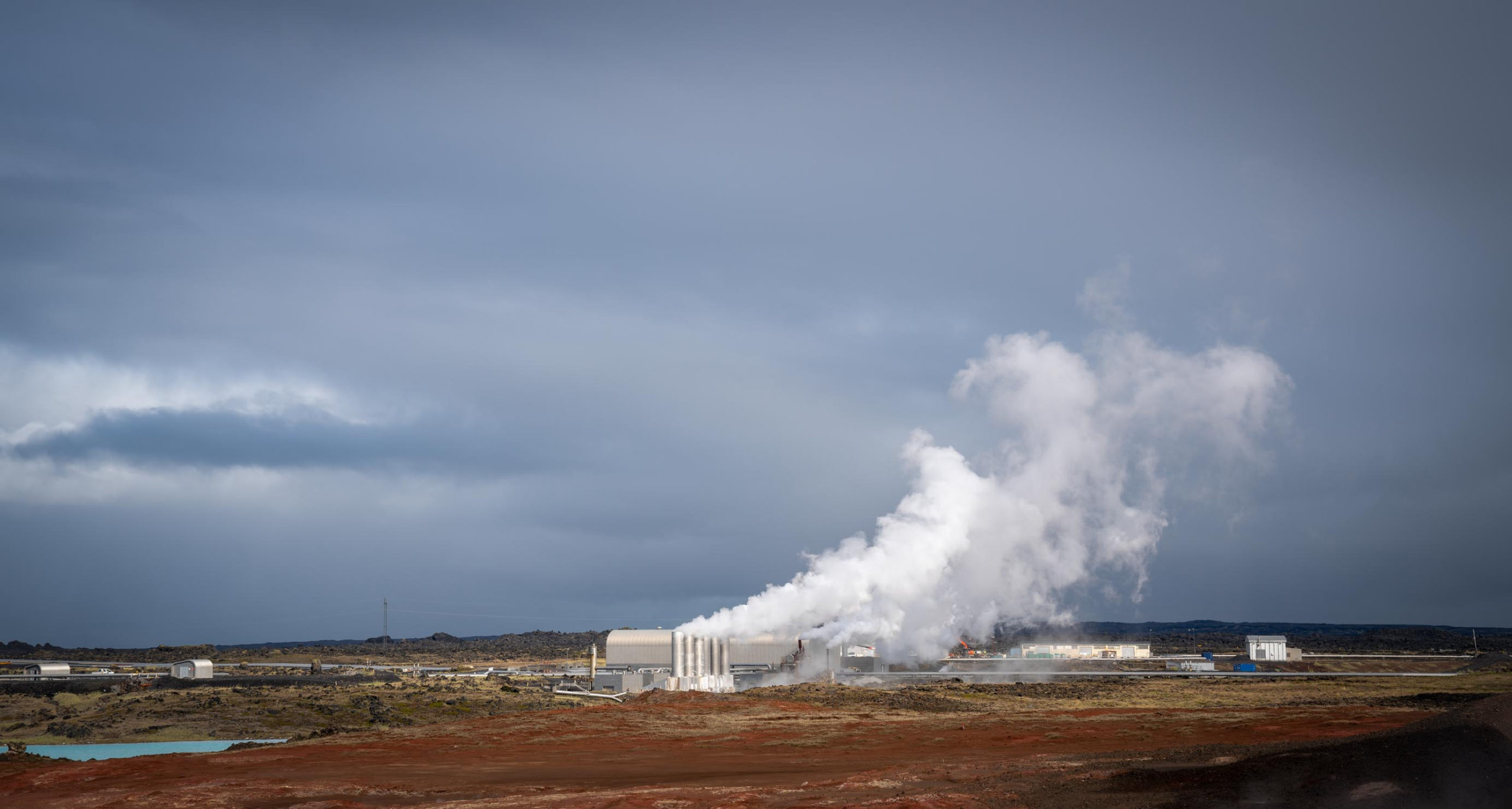
This is part of our special feature, Sustainable European Cities and Digitization.
Click here for Part II.
A wide global consensus has been reached that it’s time to foster sustainable, just, and “green” transformations of society, irrespective of our cultural backgrounds and prosperity levels. This transformation covers all parts of society, thereby influencing our footprints on Earth. European policies now follow catchwords like “decarbonization,” “circular economy,” or “social inclusiveness.” The energy sector represents a crucial part of this transformation process, inside which the heating and cooling of buildings are responsible for almost 50 percent of the final energy consumption and more than 25 percent of the overall greenhouse gas (GHG)emissions (Mathiesen et al., 2019 ). But unlike the electricity sector, the building sector has not been addressed exhaustively by politics in the past decade and is therefore lagging behind when it comes to the green and sustainable transformation. Consequently, only about 19 percent of the energy consumption for heating and cooling in Europe is currently generated by renewable energy, underlining that there is a large potential for improvements (Source: EuroStat, 2019).
This article takes a look at hidden opportunities for supporting the green and livable urban transformation by using geothermal energy for the heating and cooling of buildings. Geothermal energy is a natural (and one of the oldest) energy sources available to mankind, which has been put aside in favor of carbon-based technological developments in the past centuries. Currently, it covers a niche inside the energy sector. Natural heat provided by the Earth is available everywhere and may theoretically cover most of our energy demands for thousands of years. Talking about shifting our energy system towards green, environmentally friendly, and on-site available sources like geothermal has an impact on a multilevel scale. It is not just about GHG savings in order to mitigate global warming—it also influences higher health standards by avoiding emissions, such as aerosols or excess heat from cooling. To an even greater extent, the green transformation has strong geopolitical implications by reducing the dependency on energy imports. However, such transformation processes require multilateral technological and socio-economic efforts. This change can only be successfully accomplished if joint long-term visions prevail over short-term gains, which is even truer for the use of geothermal energy.
Urban areas are important hubs for technological and societal transformations
Urban settlements already cover 75 percent of the EU’s population and are expected to rise to a level of around 85 percent in 2050 (United Nations, 2018). It needs to be pointed out that the urban population currently already produces about 80 percent of the GHG emissions (Hoornweg et al., 2011). Urban areas are hubs for innovation and efficiency, when it comes to the use of resources and the introduction of new concepts of life. On the other hand, they are much more sensitive and vulnerable to exogenous changes of the overall living conditions.
Looking at the residential sector, the biggest challenges can be summarized as:
- Impacts of climate change regarding the so-called urban heat island effects, which means that temperatures especially in cities increasing that may induce health issues
- Impact of emissions, especially aerosols on the health conditions of urban residents, which in turn lead to reduced resilience towards respiratory diseases as currently observed during the COVID-19 pandemic
- Adaptation of existing infrastructure like buildings, public places or roads to become part of the multi-scale transformation process
- Dependency on energy imports, which affect the social stability due to volatile energy costs
- Ensuring social inclusiveness and affordability in order to leave nobody behind
Geothermal energy a hidden asset
The Earth has the ability to produce and store heat, so-called geothermal energy. The main sources of geothermal energy are represented by natural radioactivity, residual heat related to the gravitational compaction leading to the solidification of our planet as well as latent heat released by the crystallization of the Earth’s core. Our globe shows temperatures above 1000°C at 99 percent of its volume, and reaches more than 5000°C at its center. However, the natural heat loss at the surface is rather low at a global average of 70 mW/m², which goes in line with the capacity of single room heating devices (e.g. a 1 kW portable electric heater) at an area of twice a soccer field.
Nevertheless, this heat can be technically used in many different ways as geothermal energy accumulates at vast subsurface rock volumes. Depending on the depth level of geothermal heat exchangers, temperature levels between less than 10°C and up to more than 200°C or more, harvested in depth levels between a few meters and several kilometers below the surface, can be utilized for producing heat, cold or electric power. Moreover, the subsurface offers excellent opportunities to store excess heat or cold at large volumes for the next season.
There are different approaches to classify geothermal technologies. The most popular classification refers to the depth level at which the heat transfer in the Earth takes place: Shallow geothermal energy covers application using a range of the uppermost meters to several hundreds of meters of the subsurface, medium depth covers the range between around 500 and 2000 meters and deep geothermal represents heat sources at depths greater than around 2000 meters. As the Earth’s temperature increases with depth at a global average of around 30°C per kilometer, geothermal technologies can also be classified by the temperature level of the geothermal source used, which corresponds to its enthalpy. Ambient geothermal systems, mostly covered by shallow geothermal, operate at temperatures below 30°C and, in most cases, require the use of heat-pumps for heating. Low enthalpy systems mark all kinds of uses for heating purposes, which do not allow for the production of electricity by thermal processes. There is no strict boundary for the production of electricity, but most transformation technologies require source temperatures above 90°C. Moderate enthalpy systems cover geothermal sources above 90°C showing a high exergy content, which allows for generating electric power. Finally, geothermal technologies can be differentiated by the subsurface heat exchanger, which are mostly linked to boreholes. Closed loop systems represent tens to hundreds of meters deep boreholes, which host pipes in which a heat carrier fluid circuits and geothermal heat is transferred via conduction. This technology is common in shallow- to medium depth geothermal uses (borehole heat exchangers). In contrast, open loop systems have a direct connection to fluids circulating in natural or artificial pore spaces in the subsurface. This covers the use of natural subsurface fluids in depths between less than 50 meters (groundwater heat exchangers) and several kilometers (hydrogeothermal energy use). Since the 1970s, the geothermal technology portfolio has also been extended towards petrothermal energy use, which covers the engineering of artificial open loop systems in hot but no water bearing rocks by hydraulic stimulation (Hot Dry Rock or Enhanced Geothermal Systems). However, petrothermal energy use is still at the piloting phase and hydrogeothermal energy use is still a state-of-the-art technology.
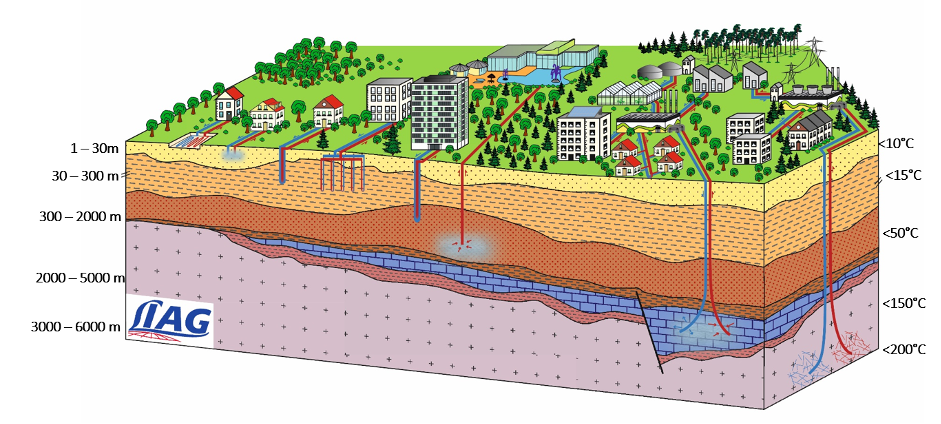
Figure 1: Overview of geothermal technologies (source: Leibniz Institute for Applied Geophysics, Hanover, Germany).
As for the year 2019, eight-eight countries worldwide are using geothermal energy in the range of 400 TWh per year for various purposes. Referring to Lund & Toth (2020), the most common and rapidly growing use is given by ambient geothermal heat pumps for space heating (44 percent of geothermal heat used), followed by electricity production (25 percent), spas (14 percent) and direct heat heating including district heating (12 percent). Nevertheless, geothermal energy still covers a niche inside the global energy sector at shares between less than 1 percent (global average) and less than 3 percent (Europe).
Geothermal energy and heating and cooling networks—a promising combination for supporting the urban green transformation
In the conventional sense, a heating network just consists of one or more heating sources (e.g. a central gas fired boiler or a geothermal heat plant), which is connected to a series of consumers via a network of pipelines. The network itself is neutral to heating and cooling sources but controlled by the temperature level and acts as “local marketplaces for energy” (Werner, 2017). District heating (DH) networks are important hubs for raising the efficiency and renewable energy share in the heating and cooling sector. Since the first DH systems were introduced in the late-nineteenth century (e.g. New York), several generations of concepts developed. While the first generation, coal fired DH systems that were based on hot pressurized steam at temperature levels up to 200°C, was affected by high energy losses and certain risks of hazards (e.g. steam explosions), the subsequent generations of networks continuously reduced the related temperature levels down to 50°C and less (4th generation). Along with the reduction of the network temperatures, the accessible heating sources could be significantly enlarged, which turned centralized monovalent DH networks into more decentralized, multivalent ones. The latest, fifth generation, mainly uses on-site available heating sources at ambient temperature levels or slightly above, which includes solar thermal, ambient geothermal or waste heat from industrial or commercial uses. Moreover, such networks may offer heating and cooling at the same time to the clients (DHC—district heating and cooling networks). In this context, clients might become both consumers and producers (e.g. dumping waste heat into the DHC network) empowering them to “prosumers.”
Looking at the current situation in Europe, district heating covers around twelve percent of the heating supply. However, the overall efficiency of the heating sector correlates with the share of DH systems (Kavvadias et al. 2019). In Europe, we can identify three different clusters referring to the use of DH systems: (1) Frontrunners like the Scandinavian and some Baltic countries, which managed to have a high share of DH systems along with a high share of renewables and a low share of fossil energy use. The concept of DH systems was introduced after the oil crises of the 1970s and therefore led to modern DH networks of generation three and later. (2) Fossil fired DH networks play a significant role in many central and eastern European countries, which were installed between the 1950s and 1980s. Due to higher temperature levels and use of fossils, both, the share of renewables as well as system efficiencies are rather low in those countries. (3) Finally, many western—as well as southern European countries did not follow the concept of DH systems and therefore show a very high share of fossil or electric energy in the heating sector, resulting in only moderate levels of renewables and energy efficiency. In order to realize the green and sustainable transformation of the heating and cooling sector, individual solutions must be found especially for cluster (2) and (3). Due to its broad technological scale, geothermal district heating and cooling networks are able to provide solutions for all the clusters mentioned above.
The main technological approach of Geothermal-DHC is based on novel district heating and cooling network typologies, which are shown in Figure 2 below and support the basic ideas of enabling DHC networks to become technologically neutral marketplaces for energy.
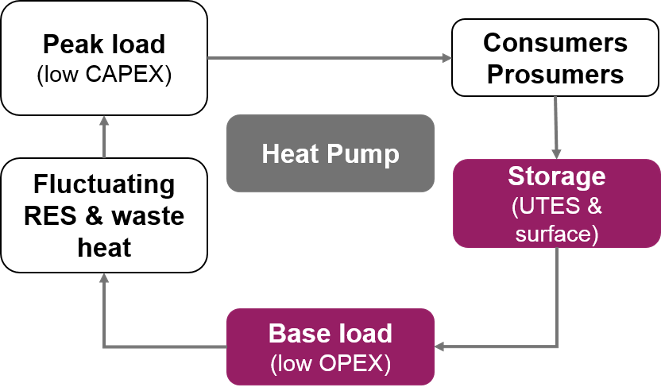
Figure 2: Modern district heating and cooling typologies integrating geothermal energy in a twofold way in terms of base load supply heating and cooling source as well as in terms of a seasonal storage (Source: Geothermal-DHC).
Modern DHC typologies, addressed by the COST Action Geothermal-DHC, use on-site available geothermal resources at different temperature levels for base load supply due to the low level of operational costs (OPEX). Peak load as well as back-up supply are realized by flexible and high enthalpy sources such as green gas or biomass, which moreover are affected by low investment costs (CAPEX). As only operating for a limited number of hours per year the OPEX related to peak load supply heating sources are not relevant. In addition, such modern DHC typologies also integrate fluctuating on-site renewable and residual heating sources, such as ambient air, solar thermal or excess heat from space cooling and commercial cooling applications. As the availability of these heating sources may strongly vary in time, storage solutions are needed for a full valorization with regard to the DHC network. For that reason, geothermal can also provide a large capacity seasonal heat and cold storage by underground thermal energy storage (UTES). UTES technologies offer storage solutions for temperature levels between less than 10°C and up to 90°C using either the solid or subsurface or groundwater filled pore spaces. For small scale applications at storage capacities below 1 GWh heat per year and storage temperature levels below 30°C, UTES has already reached market readiness. Larger UTES systems at higher temperature are currently in the demonstration and piloting phase in Europe. Heat pumps, operating either centralized or decentralized at different capacity and temperature levels complement the modern DHC typologies as they are acting as “moderators of energy market places” by modulating the different temperature levels between heat and cold sources supplying the DHC network on the one hand and the different temperature level requirements of the network consumers on the other hand. In general, such typologies can be applied to new as well as to existing district heating and cooling networks of different technological generations.
Geothermal cooling—an emerging concept to mitigate urban heat islands
Climate change induces rising temperatures; further, building density intensifies the heat island effect in cities. Together with growing expectations by residents and employees concerning their thermal wellbeing, the cooling demand within cities is expected to significantly increase during the next decades. To satisfy this growing cooling demand, the most common application used is the decentralized vapor compression cycle (VCC). The VCC circulates a refrigerant, which absorbs and removes heat from the building that needs to be cooled and then rejects the heat to the environment. Thus, while this concept has been well established for decades and is highly reliable, it is also characterized by a high demand for electrical energy as well as local emitted excess heat, which might further increase the heat island effect.Geothermal energy may be a promising alternative for meeting the increasing cooling demand in an environment-friendly way. There are different technical concepts for using geothermal energy in order to provide cooling. In case of medium or deep geothermal energy with a heat source temperature above 70°C, absorption chillers are an attractive solution for providing cooling. Any shallow geothermal system can be used for cooling either by operating a reversible heat pump or by direct cooling. Especially the direct use of groundwater provides a very efficient way of cooling. For that purpose the cold stored in groundwater is transferred via wells and heat exchangers to the building. The required electricity demand for those systems is significantly smaller compared with the common VCC concepts.
Brave visions are needed to enable the green transformation
The EU funded project Heat Roadmap Europe concluded that a full decarbonization of the heating and cooling sector can be achieved by 2050 with already existing technologies and without a massive expansion of bioenergy use. Future district heating and cooling grids could cover up to 50 percent of the heat demand. By 2023, the COST Action Geothermal-DHC will elaborate a roadmap towards a better integration of geothermally-supplied heating and cooling networks in Europe. The 2030 goals is to shift 1) the share of DH networks in the heating and cooling sector from twelve to thirty percent and 2) to shift the share of geothermal energy inside DH networks from currently less than 3 percent to above 10 percent. By 2050, these shifts should reach fifty percent in both cases.
The transformation of the heating and cooling sector demands fundamental shifts of paradigms and strategies, especially in urban areas. One possible future approach is covered by the principle of exergy prioritization, as shown in Figure 3. The key idea is to better match temperature levels, which reflects exergy levels, between the heat demand and the heat source. Space conditioning is responsible for almost 25 percent of all end energy demands in Europe and requires temperature levels between less than 20°C for space cooling and up to 70°C for hot domestic water supply or conventional heat distribution radiators. Exergetic prioritization would privilege low temperature heating sources like ambient heat, geothermal or solar thermal to cover space conditioning while high exergy related heating sources, such as green gas or electricity are reserved for industrial processes.
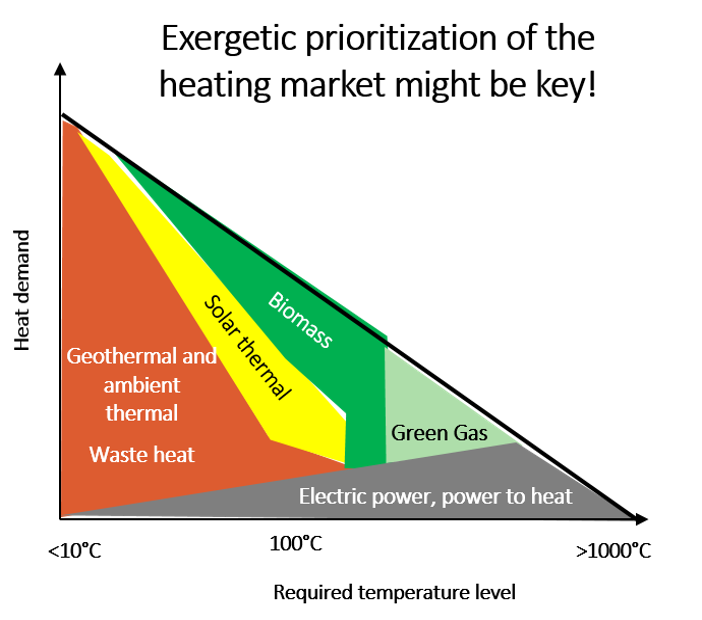
Figure 3: The concept of exergetic prioritization on the heating market—matching the temperature levels of heat demand and heat supply (source: Geothermal-DHC).
The European Geothermal Energy Council (EGEC) recently proclaimed that the next decade until 2030 will become the “geothermal decade”—it is now up to all actors in the energy market to make this vision reality.
Gregor Goetzl, MSc, is a senior researcher on geothermal energy use at the Geological Survey of Austria. Since 2019, he is chairing the EU COST Action CA18219 Geothermal-DHC, which focuses on the integration of geothermal energy into heating and cooling networks.
Kai Zosseder, Dr., is Head of the Geothermal Group of the Chair of Hydrogeology at the Technical University of Munich. He has been working since 2010 in the field of Geothermal Energy, leads many projects concerning Geothermal Energy Developments and is Executive Member of the EU COST Action CA18219 Geothermal-DHC.
Ana Vranjes, Dr., is an Assistant professor in the Department of Hydrogeology at the Faculty of Mining and Geology, University of Belgrade. She is a leading researcher in the field of geothermal energy at the UoB, actively involved in numerous national and international geothermal projects, including EU COST Action CA18219 Geothermal-DHC.
Christopher Schifflechner, MSc, is a researcher and PhD student at the Chair of Energy Systems at the Technical University of Munich. His research focuses on flexible and efficient combined heating, cooling and power generation from deep geothermal resources.
Jessica Chicco, Dr., is a Post Doc at the University of Turin (Italy). She is working on different projects concerning low enthalpy geothermal energy for direct uses as well as underground thermal energy storage systems. She is currently involved in the EU COST Action CA18219 Geothermal-DHC as co-leader of PWG1 about Technology, and Leader of the Ad-Hoc Working Group on underground storage systems.
Rao Martand Singh, Dr., is a Professor in the Department of Civil & Environmental Engineering at NTNU (Norwegian University of Science & Technology). He has been working in the area of energy geotechnics for last 12 years, mainly focusing on geothermal energy structures such as energy piles and energy tunnels. He is the Executive Member of the EU COST Action CA18219 Geothermal-DHC.
This article reflects the work of the currently operating COST Actions CA18219 Geothermal-DHC. COST is a European research funding program under the Horizon 2020 framework to support the promotion of scientific excellence. For more information please also visit www.geothermal-dhc.eu.
Click here for Part II.
References
Della Vedova B., Cimolino. A., Castelli E., and Brancatelli G. (2015). Geothermal Heating and Cooling in the FVG Region: the Grado District Heating and the Pontebba Ice Rink Plants. Proceedings and AdrJo.Platform, Losinj 2014 Workshop on Geothermal Energy, pp. 65-76.
EU Commission, The European Green Deal (2019), COM(2019)640, Brussels.
Hoornweg, D., Sugar, L., Trejos Gomez, C. T. (2011) Cities and greenhouse gas emissions: moving forward. Environment & Urbanization, 23(1), 207–227. https://doi.org/10.1177 percent2F0956247810392270
Kavvadias K., Jiménez-Navarro J.P., Thomassen G., Decarbonising the EU heating sector – Integration of the power and heating sector, EUR 29772 EN, Publications Office of the European Union, Luxembourg, 2019, ISBN 978-92-76-08386-3, doi:10.2760/943257, JRC114758
Lund J.W, Toth A. N. (2020): Direct Utilization of Geothermal Energy 2020 Worldwide Review, Proceedings World Geothermal Congress 2020 Reykjavik, Iceland, April 26 –May 2, 2020.
Manente G., Lazzaretto A., Molinari I.,and Bronzini F. (2019). Optimization of the hydraulic performance and integration of a heat storage in the geothermal and waste-to-energy district heating system of Ferrara, Journal of Cleaner Production, Volume 230, pp 869-887, ISSN 0959-6526, https://doi.org/10.1016/j.jclepro.2019.05.146.
Mathiesen B.V., Bertelsen N., Schneider N.C.A., Garcia Sanchez L., Paardekooper S., Thellufsen J.Z., Djorup S.R., Towards a decarbonized heating and cooling sector in Europe: Unlocking the potential of energy efficiency and district energy (2019, Aalborg Universitet, Denmark.
United Nations, Department of Economic and Social Affairs, Population Division (2018). World Urbanization Prospects: The 2018 Revision, Online Edition.
Werner S, International review of district heating and cooling (2017), Energy 137 (2017) pp. 617 – 631, Elsevier.
Photo: Steaming geothermal hot water, Hveragerdi, Iceland | Shutterstock
Published on May 11, 2021.