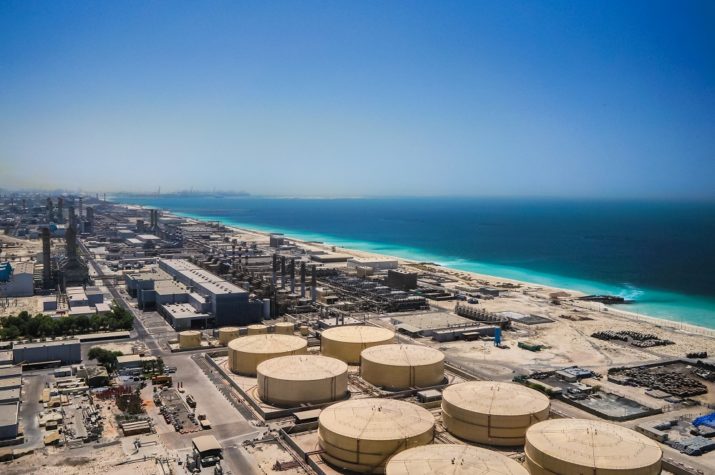
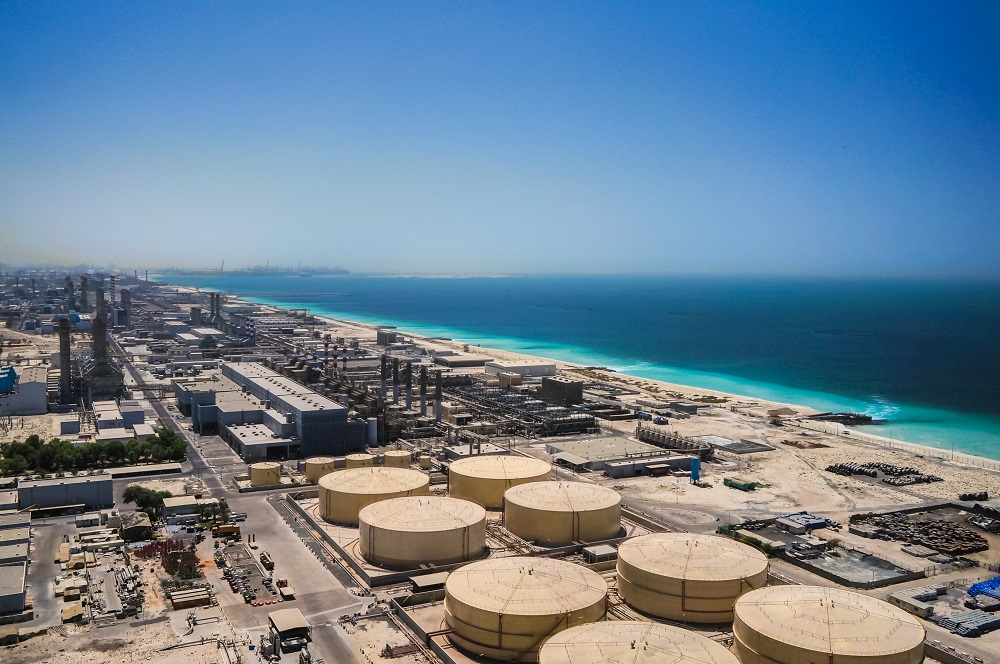
This is part of our special feature on Water in Europe and the World.
Within the next decade, water shortages are projected to affect 40 US states and effectively all Americans.1 The issue of water accessibility is not one limited to the US, however, as the problem of clean water availability has become more widely recognized in recent years. For example, the US National Academy of Engineering has recognized the urgent need to provide access to clean water as one of the “Grand Challenges for Engineering.”2 Desalination, or the separation of salt from water, will play a key role in addressing this challenge, as the vast majority of water sources on Earth are too salty to be used for agricultural or domestic purposes.3
Coupled to this need to provide access to clean water is the need to do so in an energy efficient and environmentally friendly manner. The intrinsic coupling between water and energy resources has been recognized broadly over the last decade.4-10 Ultimately, there is a fundamental energy cost associated with purifying water, and many widely used energy generation technologies require clean water (e.g., for cooling and/or to operate boilers). For example, in the United States, more than 133 billion gallons of water per day (roughly 41 percent of the total freshwater and saline-water withdrawal) is used for thermo-electric power generation.11 Furthermore, the laws of thermodynamics set the required minimum energy for desalination; 1.06 kWh is required to produce one cubic meter of desalinated water from two cubic meters of seawater containing a “standard” amount of salt (35 grams of sodium chloride per liter).12 This energy requirement, which is slightly less than the amount of energy required to operate a modern household refrigerator for a day (typically around 1.5 kWh), is simply the minimum energy required to separate the salt molecules from the water molecules and does not account for inefficiency in the process or other operating expenses associated with the desalination process. Desalination will always involve an energetic cost, but improvements in efficiency and process design over the last few decades have pushed desalination technology much closer to the fundamental limit for the energy cost of the overall process.12
Desalination technology is neither new nor perfect. Several technologies currently are used to desalinate water, and a combination of different challenges and application needs creates a situation where no single technology is a perfect fit for all situations. Desalination is performed on a very large scale, and the volume of water desalinated worldwide each day exceeds the volume of oil produced worldwide each day by roughly a factor of six.13-14 Let me discuss the common desalination technologies and technological challenges.
What is desalination and how is it done?
Desalination generally refers to any process that separates salt and water. Often, the salty water in question broadly can be characterized as either seawater or brackish water, depending on the salinity of the water. The salt content of water varies depending on the source, but seawater typically has a total dissolved solids, TDS, (or salt) concentration of 35 to 45 grams per liter, while brackish water sources typically have TDS concentrations of 1 to 10 grams per liter.15 The specific desalination process used typically depends on the salt concentration of the water source as well as other factors, such as the specific contaminants found in a given water source.
Desalination technologies often fall into two broad classes: thermally-driven or barrier desalination processes. Thermally-driven processes remove water from salt by boiling or otherwise vaporizing water; salt is not volatile, so water can effectively be removed from salt by this mechanism.16 Barrier methods typically involve a selective membrane to perform the separation; in processes such as reverse osmosis (RO) and nanofiltration (NF), water selectively passes through the membrane leaving the salt behind (Figure 1A), but in electrodialysis (ED) processes, electricity is used to drive selective transport of charged salt ions through special charged membranes, effectively removing the salt from the water (Figure 1B).17
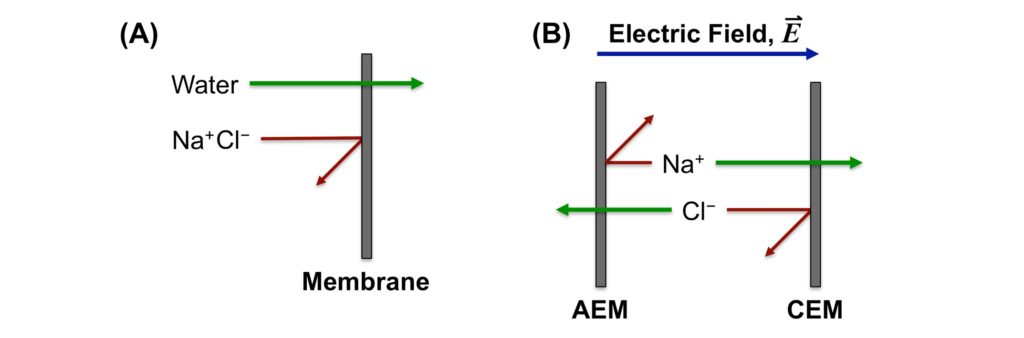
Figure 1. (A) In reverse osmosis (RO) or nanofiltration (NF) desalination, water selectively passes through the membrane leaving salt (e.g., sodium chloride, NaCl) behind. (B) In electrodialysis (ED) desalination, ions are moved through special anion exchange or cation exchange membranes (AEMs or CEMs).
In general, ED processes are most efficient in situations where the source water is not particularly saline, and RO processes are used more widely for desalinating higher salinity water.17 If the salinity is too high, however, thermally-driven desalination methods can become the most efficient desalination method.17 For particularly hard water sources, NF desalination can be used to selectively remove the calcium and magnesium ions that contribute to water hardness.17
Reverse osmosis technology currently dominates the desalination market, and more than half of the worldwide installed desalination capacity is based on RO technology (Figure 2).16 This situation is largely driven by the low economic and energy costs of RO technology compared to thermally-driven multi-stage flash (MSF) and multi-effect distillation (MED) processes or other technologies.16, 18 The majority of installed desalination capacity is located in the Middle East, where seawater is plentiful and freshwater is sparse, but desalination is routinely performed around the world with significant growth, over the last decade, in the Mediterranean, Asia, and the Americas.19
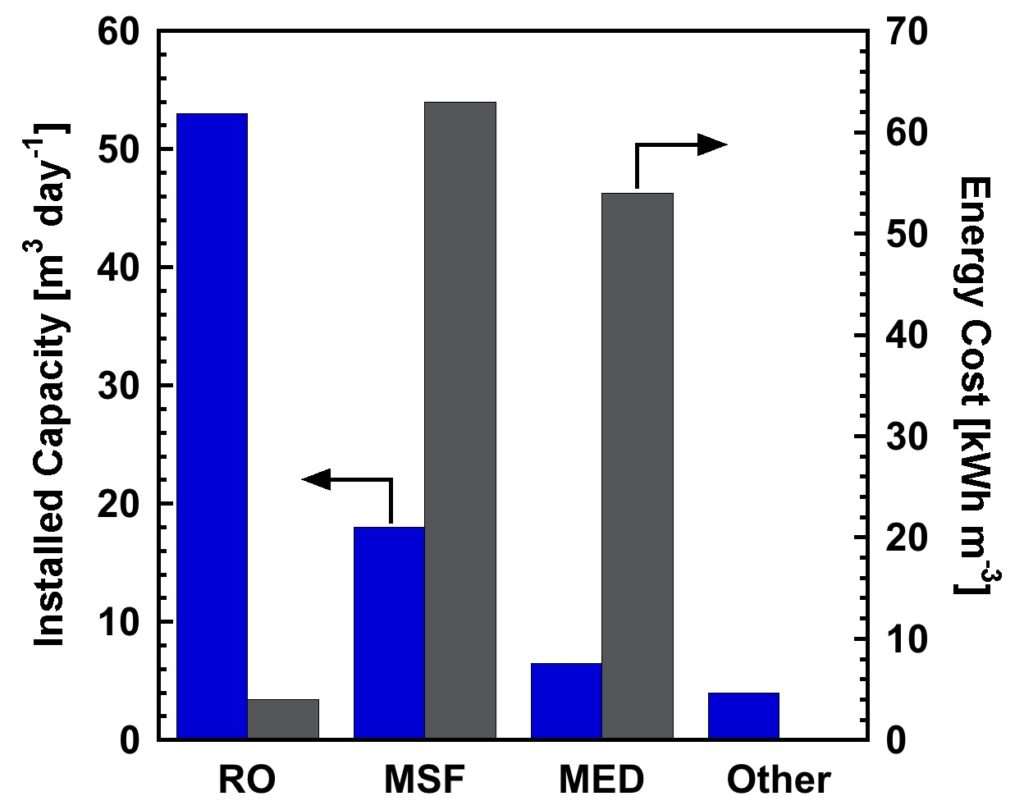
Figure 2. Worldwide installed desalination capacity and average energy costs for seawater desalination using reverse osmosis (RO), thermally-driven processes: multi-stage flash (MSF) and multi-effect distillation (MED), and other technologies.16, 18
The energy cost of seawater RO desalination has decreased by roughly a factor of nine since 1970.12 Some of this improvement has resulted from improvements in desalination membranes. The majority, however, of the improvement in efficiency resulted from the development and implementation of pressure exchangers that are able to recover pressure (mechanical energy) that would otherwise be lost, as the concentrate (i.e., water that was not purified) at the end of the process often leaves the membrane module at a pressure of around 400 psi (27.5 bar) or greater.20
Technological Needs for the Future of Desalination
Pipe Parity. While the economic and energy costs of desalination have dropped significantly over recent decades, the total cost of producing water is still too high to be accessible to many communities.8 As such, technological improvements are needed to reduce the cost of producing water via new technologies. This goal is embodied in the push toward achieving “pipe parity” whereby purified water could be produced with equivalent economic, energy, and carbon costs compared to that required to provide water from natural sources.8 This target, outlined by the White House in 2015, requires significant reductions in water costs resulting from operating, capital, energy, system integration, and other soft costs associated with the desalination process. Altogether, achieving pipe parity would likely require a reduction in water cost from approximately $2.00 per cubic meter of purified water to $0.50 per cubic meter of purified water.7-9
Technological improvements will play an important role in the push toward pipe parity, as improved desalination membrane technology, for example, could reduce both capital and operating costs in RO plants. While membranes make up a sizable portion of the capital costs of a desalination plant, operating and cleaning membranes accounts for a significant portion of desalination operating expenses.8 Membranes that resist fouling and offer low resistance to water flow (without compromising selectivity) could considerably reduce the operating expenses of desalination.21 Additionally, integrating emerging desalination and energy recovery processes into desalination systems could further improve the economics of desalination and push the overall efficiency toward the pipe parity targets.8, 16
Considerable worldwide research effort is directed toward engineering new membranes that would contribute to achieving these goals. Addressing the membrane fouling / cleanability issue can be approached both from the perspective of modifying membrane chemistry (or applying anti-fouling coatings to membranes) or engineering the membrane modules themselves to more effectively mitigate the collection and buildup of biological and organic foulants.22 The vast spectrum of foulants and the adaptable nature of biology, however, make it difficult to broadly realize this goal. Addressing the challenge of preparing membranes that offer low resistance to water flow is also an active area of research, and emerging nanocomposite and bio-inspired membranes are becoming viable and other promising technologies and approaches are in the pipeline (though likely years away from industrial viability).23-24
Desalination and Renewable/Clean Energy
Due to the extremely large scale of desalination, a considerable amount of energy is needed to purify large amounts of water.8 Desalination plants often rely on traditional non-renewable fossil fuel energy sources, so the process of desalination ends up having a considerable carbon footprint.25 One way to address this issue is to integrate renewable energy sources and desalination processes.26 While this goal could be accomplished by using renewable energy sources to power conventional desalination plants, interest also exists in leveraging solar energy to directly desalinate water. Concentrated solar energy (i.e., sunlight focused by lenses and/or mirrors) can be used to do desalination by focusing solar radiation into a receiver that collects energy from the sun as heat; this heat can then be used to desalinate water by evaporating pure water from a saline source.25
The energy cost of desalination can be offset, to some extent, by coupling emerging renewable energy technologies, such as pressure retarded osmosis (PRO) or reverse electrodialysis (RED), with conventional desalination processes.23, 25 The PRO and RED technologies can harvest some of the chemical energy that is present in the highly concentrated brine that is left over from the RO.3 If managed properly, this type of integrated process can lead to an overall reduction in the energy required to desalinate water.
Point of Use Desalination Systems
In regions of the world where municipal water infrastructure is not as well developed or accessible, point of use desalination systems (i.e., home or under-sink water purifiers) could be a solution.27 In 2017, a Chinese standard (GB 34914-2017) was released to grade or rate the efficiency of this class of water purifiers.28 The standard outlines efficiency grades that correspond to water production rate achieved using standardized feed conditions and purity specifications.
A notable aspect of the standard is that the water used in the test is a mixture of salts (in comparison to the conventional method of testing desalination membranes using only table salt). The standard requires the use of 278 parts per million (ppm) calcium chloride (a salt that is often used in foods and drinks to provide salty taste without adding sodium), 269 ppm sodium bicarbonate (baking soda), and 50 ppm sodium chloride (table salt) in water.28 Desalination membrane performance long has been evaluated using sodium chloride (i.e., table salt), as historically, sodium chloride was the most challenging salt to remove during RO membrane desalination.29 As purification standards have become more strict, manufactures increasingly need to know how well desalination membranes perform while desalinating water that contains more than simply table salt. As such, the GB 34914-2017 standard takes a step toward requiring a different type of mixed salt characterization of desalination membrane performance. This standard will likely influence the development of new membrane materials for this emerging point of use water purifier market.
My research focuses on issues related to this challenge. We seek to understand why desalination membrane performance changes when membranes are contacted with salts that are different from table salt and/or are contacted with mixtures of different salts. By understanding these changes, we seek to engineer advanced membranes that desalinate water, containing multiple salts, more effectively and efficiently than the current state-of-the-art.
Zero- (or minimal-) Liquid Discharge
Most desalination plants do not purify all of the water fed to the plant, so plants often need to dispose a concentrated brine solution that is leftover after the process. Disposal can be expensive, particularly for inland plants, as the brine can have negative environmental impacts.30 As such, interest exists in pursuing aggressive zero-liquid (or minimal-liquid) discharge strategies for desalination.
These strategies aim to reduce the amount of waste liquid (i.e., brine) that is discharged following desalination. Often while doing so, it may be possible to recover salts that could be sold to recover some of the economic cost of the process. While historically viewed as excessively expensive, environmental concerns and regulatory environments have renewed interest in exploring avenues for minimizing the amount of brine that is released as a byproduct of desalination processes.30
Hybrid processes are often used to press toward this goal of minimizing or eliminating liquid discharge from desalination plants. While RO can be used to concentrate the brine, reductions in efficiency and potential damage to components due to mineral scaling limit how much the brine can be concentrated using RO.30 Following RO, electrodialysis (ED), forward osmosis (FO), or membrane distillation (MD) could be used to further concentrate the brine before ultimately eliminating the remaining liquid by crystallizing salt in an evaporation pond or a dedicated crystallizer.30
Recovery of Nutrients or Valuable Contaminants
While working to minimize liquid discharge from water treatment plants, salts and/or other contaminants that may be of value could be recovered. Recent political developments may affect the supply of rare earth elements, which are needed for a number of technological applications,31 so opportunities to recover these materials from water could become a viable strategy.32 Additionally, the energy required to make fertilizer has generated interest in recovering nutrients from groundwater (particularly in agricultural areas where runoff contamination also threatens drinking water sources).33 A critical challenge in this area is engineering the separations to fractionate different ionic compounds, as most of these contaminants are present in water at very dilute concentration, and this area of research is also a focus of my research efforts.
Public Perceptions and the Implementation of Desalination Plants
Water scarcity is expected to continue to increase, so demand for purified water likely will increase in the future. Seawater desalination is poised to play an increasingly important role in meeting increasing demand for purified water. Seawater desalination plants are often necessarily located in coastal regions, and public perception is an important factor that will influence the installation of future desalination capacity. Public perception about desalination to produce drinking water is generally favorable (based on studies conducted in regions of California and Australia), but environmental and plant location concerns are important issues that affect public perceptions about and acceptance of desalination.34
The growth of coastal populations and the desire to build desalination capacity near the ocean suggest that seawater desalination processes could effectively increase drinking water supplies to meet demand, but it also introduces challenges in terms of constructing new desalination plants in a manner that aligns with public opinion, perception, and priorities.22, 34 Seawater desalination plants must be integrated into the local ecosystem, and to maintain favorable public perception and acceptance of desalination, impact on the ecosystem must be minimized where possible.34
Seawater desalination plants extract water from the ocean and then return a concentrated brine to the ocean during normal operation. These activities can disrupt ecosystems and marine organisms, and while strategies (such as distributed piping) can reduce this impact, it is important to communicate these strategies to the public.34 Additionally, coastal plant locations can introduce problems as many residents recognize the value of desalination but do not necessarily want to live near a plant. In some areas, construction of desalination plants in industrial coastal areas may not be as much of a barrier, but as land becomes more scarce and desalination capacity is increasingly needed, these issues may become more significant.34
Additionally, providing water via seawater desalination can be expensive, as plant construction requires significant capital investment and water costs are higher compared to potable water derived from many other freshwater sources. As such, public perception is important because public support of the financial model for desalination can influence the adoption of desalination strategies. For example, in 2013, the desalination plant in Torrevieja, Spain, one of the largest desalination plants constructed in Europe, sat idle six years after construction began because people were not willing to pay for the water and the government was not able to subsidize drinking water costs to make desalination viable.35 Five years later, however, government investment could help to expand and triple the water output of the plant, which was operating at just over 50 percent of capacity in March 2018.36 The situation in Torrevieja illustrates a complex interplay of demand for water, the cost of water infrastructure, public perception / willingness to pay for drinking water, and politics that likely will continue to affect future implementation of desalination around the globe.
Outlook for Desalination
Growing demand for water resources will continue to create demand for expanded desalination capacity worldwide. As this development occurs, realization of pipe parity and zero/minimal liquid discharge targets will continue to push the technology forward. Additionally, emerging regulations should encourage membrane manufacturers to design membranes for relevant feedwaters, and the opportunity to recover nutrients and/or other valuable contaminants may help to offset desalination costs. Improvements in membranes and system design (including integration of renewable energy sources and/or generation) will be critical for reducing the overall economic and energetic costs of desalination, and new desalination capacity will need to be implemented in a manner that is sensitive to public perception.
Geoffrey M. Geise is an assistant professor of chemical engineering at the University of Virginia. After earning a B.S. degree in chemical engineering from the Pennsylvania State University in 2007, he proceeded to earn M.S.E. (2010) and Ph.D. (2012) degrees in chemical engineering from the University of Texas at Austin where he developed experimental techniques for measuring individual ion sorption in polymers and established a fundamental selectivity/permeability tradeoff relationship in desalination membrane materials. At the University of Virginia, his research focuses on studying the fundamentals of chemically- and electrochemically-driven small molecule transport through polymeric materials in order to engineer membranes that will address global water shortages and need for clean energy. He has received several professional and academic awards and honors including the NSF CAREER Award, 2016 Ralph E. Powe Junior Faculty award, the 2015 Young Membrane Scientist Award from the North American Membrane Society (NAMS), the New Professor Travel Award from Engineering Conferences International, and a University of Virginia Excellence in Diversity Fellowship. He is affiliated with the Global Water Initiative
References:
- Freshwater: Supply concerns continue, and uncertainties complicate planning, GAO-14-430; United States Government Accountability Office: May 20, 2014.
- NAE, Grand challenges for engineering: Provide access to clean water. http://www.engineeringchallenges.org/challenges/water.aspx (accessed October 2018).
- Geise; Lee; Miller; Freeman; McGrath; Paul, J. Polym. Sci., Part B: Polym. Phys. 2010, 48, 1685.
- The water-energy nexus: Challenges and opportunities; U.S. Department of Energy: June 2014.
- Energy demands on water resources: Report to Congress on the interdependency of energy and water; U.S. Department of Energy: December, 2006.
- Promoting technology innovation for clean and safe water: Water technology innovation blueprint—Version 2; EPA 820-R-14-006; U.S. Environmental Protection Agency, Office of Water: April, 2014.
- Korte; James, White House launches ‘moonshot for water’. USA Today December 15, 2015.
- The White House Water resource challenges and opportunities for water technology innovation; 2015.
- White House Water Summit: March 22, 2016.
- Food, energy, and water: Transformative research opportunities in the mathematical and physical sciences; Directorate for Mathematical Physical Sciences Advisory Committee, The National Science Foundation. https://nsf.gov/mps/advisory/mpsac_other_reports/subcommittee_report_food_water_energy_nexus_final.pdf (accessed October 2018).
- Dieter; Maupin; Caldwell; Harris; Ivahnenko; Lovelace; Barber; Linsey Estimated use of water in the United States in 2015; U.S. Geological Survey Circular 1441: 2018.
- Elimelech; Phillip, Science 2011, 333, 712.
- International Desalination Association., Desalination by the numbers. http://idadesal.org/desalination-101/desalination-by-the-numbers/ (accessed October 2018).
- The world factbook 2013-14. Central Intelligence Agency: Washington, DC, 2013.
- Greenlee; Lawler; Freeman; Marrot; Moulin, Water Res. 2009, 43, 2317.
- Miller; Shemer; Semiat, Desalination 2015, 366, 2.
- Baker, Membrane technology and applications. 3rd ed.; Wiley: New York, 2012.
- Liu; Hejazi; Kyle; Kim; Davies; Miralles; Teuling; He; Niyogi, Environ. Sci. Technol. 2016, 50, 9736.
- Fritzmann; Löwenberg; Wintgens; Melin, Desalination 2007, 216, 1.
- Stover, Desalination 2007, 203, 168.
- Cohen; Semiat; Rahardianto, AlChE J. 2017, 63, 1771.
- Fane; Wang; Hu, Angew. Chem. Int. Ed. 2015, 54, 3368.
- Amy; Ghaffour; Li; Francis; Linares; Missimer; Lattemann, Desalination 2017, 401, 16.
- McGinnis; Reimund; Ren; Xia; Chowdhury; Sun; Abril; Moon; Merrick; Park; Stevens; McCutcheon; Freeman, Science Advances 2018, 4.
- Ghaffour; Bundschuh; Mahmoudi; Goosen, Desalination 2015, 356, 94.
- Ali; Tufa; Macedonio; Curcio; Drioli, Renewable and Sustainable Energy Reviews 2018, 81, 1.
- Guidelines for drinking-water quality: Second addendum; World Health Organization: Geneva, 2008.
- Minimum allowable value of water efficiency and water efficiency grades for reverse osmosis drinking water purifiers, GB 34914-2017. General Administration of Quality Supervision, Inspection and Quarantine of the People’s Republic of China: 2017.
- Ji; Luo; Geise, J. Membr. Sci. 2018, 563, 492.
- Tong; Elimelech, Environ. Sci. Technol. 2016, 50, 6846.
- Kramer, Phys. Today 2018, 71, 22.
- Chen; Wu; Dong; Meng; Li; Yan; Chen, Sep. Purif. Technol. 2018, 197, 70.
- Jensen; Darby; Seidel; Gorman, Drinking water treatment for nitrate. Technical Report 6. In Addressing nitrate in California’s drinking water with a focus on Tulare Lake Basin and Salinas Valley groundwater. Report for the State Water Resources Control Board report to the legislature, Center for Watershed Sciences, University of California, Davis., 2012.
- Haddad; Heck; Paytan; Potts, Social issues and public acceptance of seawater desalination plants. In Sustainable Desalination Handbook, Gude, V. G., Ed. Elsevier: Cambridge, MA, 2018.
- Cala, Spain’s desalination ambitions unravel. The New York Times Oct. 9, 2013.
- Freyberg, Torrevieja desalination plant production could be trebled in Spain. Water & Wastewater International March 29, 2018.
Photo: Dubai. In the summer of 2016. Modern desalination plant on the shores of the Arabian Gulf | Shutterstock
Published on December 11, 2018.